A day in the life of the spliceosome
One of the most amazing findings in molecular biology was the discovery that eukaryotic genes are discontinuous, with coding DNA being interrupted by stretches of non-coding sequence. The subsequent realization that the intervening regions are removed from pre-mRNA transcripts via the activity of a common set of small nuclear RNAs (snRNAs), which assemble together with associated proteins into a complex known as the spliceosome, was equally surprising. How do cells coordinate the assembly of this molecular machine? And how does the spliceosome accurately recognize exons and introns to carry out the splicing reaction? Insights into these questions have been gained by studying the life cycle of spliceosomal snRNAs from their transcription, nuclear export and re-import to their dynamic assembly into the spliceosome. This assembly process can also affect the regulation of alternative splicing and has implications for human disease.
This is a preview of subscription content, access via your institution
Access options
Subscribe to this journal
Receive 12 print issues and online access
206,07 € per year
only 17,17 € per issue
Buy this article
- Purchase on SpringerLink
- Instant access to full article PDF
Prices may be subject to local taxes which are calculated during checkout
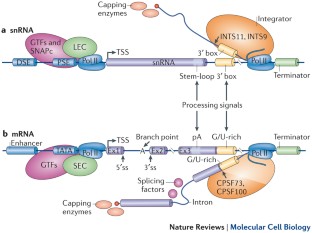
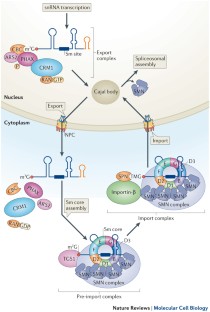
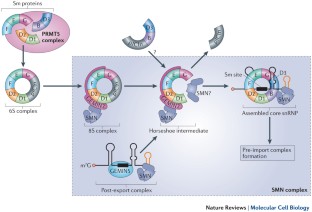
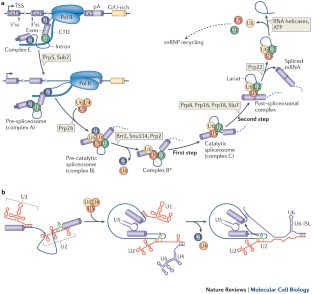
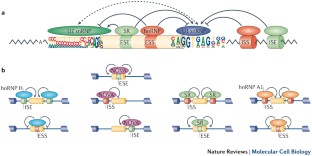
Similar content being viewed by others
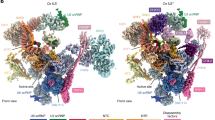
Mechanism for the initiation of spliceosome disassembly
Article 26 June 2024
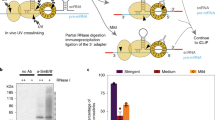
A systems view of spliceosomal assembly and branchpoints with iCLIP
Article 30 September 2019
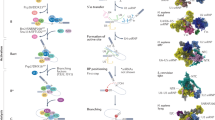
Regulation of pre-mRNA splicing: roles in physiology and disease, and therapeutic prospects
Article 16 December 2022
Change history
References
- Berget, S. M., Moore, C. & Sharp, P. A. Spliced segments at the 5′ terminus of adenovirus 2 late mRNA. Proc. Natl Acad. Sci. USA74, 3171–3175 (1977). ArticleCASPubMedPubMed CentralGoogle Scholar
- Chow, L. T., Gelinas, R. E., Broker, T. R. & Roberts, R. J. An amazing sequence arrangement at the 5′ ends of adenovirus 2 messenger RNA. Cell12, 1–8 (1977). ArticleCASPubMedGoogle Scholar
- Lerner, M. R., Boyle, J. A., Mount, S. M., Wolin, S. L. & Steitz, J. A. Are snRNPs involved in splicing? Nature283, 220–224 (1980). ArticleCASPubMedGoogle Scholar
- Will, C. L. & Luhrmann, R. Spliceosome structure and function. Cold Spring Harb. Perspect. Biol.3, a003707 (2011). ArticleCASPubMedPubMed CentralGoogle Scholar
- Jurica, M. S. & Moore, M. J. Pre-mRNA splicing: awash in a sea of proteins. Mol. Cell12, 5–14 (2003). ArticleCASPubMedGoogle Scholar
- Matera, A. G., Terns, R. M. & Terns, M. P. Non-coding RNAs: lessons from the small nuclear and small nucleolar RNAs. Nature Rev. Mol. Cell Biol.8, 209–220 (2007). ArticleCASGoogle Scholar
- Henry, R. W., Mittal, V., Ma, B., Kobayashi, R. & Hernandez, N. SNAP19 mediates the assembly of a functional core promoter complex (SNAPc) shared by RNA polymerases II and III. Genes Dev.12, 2664–2672 (1998). ArticleCASPubMedPubMed CentralGoogle Scholar
- Hung, K. H. & Stumph, W. E. Regulation of snRNA gene expression by the Drosophila melanogaster small nuclear RNA activating protein complex (DmSNAPc). Crit. Rev. Biochem. Mol. Biol.46, 11–26 (2011). ArticleCASPubMedGoogle Scholar
- Hernandez, N. & Weiner, A. M. Formation of the 3′ end of U1 snRNA requires compatible snRNA promoter elements. Cell47, 249–258 (1986). ArticleCASPubMedGoogle Scholar
- Egloff, S. et al. The integrator complex recognizes a new double mark on the RNA polymerase II carboxyl-terminal domain. J. Biol. Chem.285, 20564–20569 (2010). ArticleCASPubMedPubMed CentralGoogle Scholar
- Egloff, S. et al. Serine-7 of the RNA polymerase II CTD is specifically required for snRNA gene expression. Science318, 1777–1779 (2007). ArticleCASPubMedPubMed CentralGoogle Scholar
- Baillat, D. et al. Integrator, a multiprotein mediator of small nuclear RNA processing, associates with the C-terminal repeat of RNA polymerase II. Cell123, 265–276 (2005). Identifies the complex that carries out pre-snRNA 3′-end processing.ArticleCASPubMedGoogle Scholar
- Chen, J. et al. An RNAi screen identifies additional members of the Drosophila Integrator complex and a requirement for cyclin C/Cdk8 in snRNA 3′-end formation. RNA18, 2148–2156 (2012). ArticleCASPubMedPubMed CentralGoogle Scholar
- Weiner, A. M. E Pluribus Unum: 3′ end formation of polyadenylated mRNAs, histone mRNAs, and U snRNAs. Mol. Cell20, 168–170 (2005). ArticleCASPubMedGoogle Scholar
- Mandel, C. R. et al. Polyadenylation factor CPSF-73 is the pre-mRNA 3′-end-processing endonuclease. Nature444, 953–956 (2006). ArticleCASPubMedGoogle Scholar
- Ezzeddine, N. et al. A subset of Drosophila integrator proteins is essential for efficient U7 snRNA and spliceosomal snRNA 3′-end formation. Mol. Cell. Biol.31, 328–341 (2011). ArticleCASPubMedGoogle Scholar
- Boon, K. L. et al. prp8 mutations that cause human retinitis pigmentosa lead to a U5 snRNP maturation defect in yeast. Nature Struct. Mol. Biol.14, 1077–1083 (2007). ArticleCASGoogle Scholar
- Murphy, M. W., Olson, B. L. & Siliciano, P. G. The yeast splicing factor Prp40p contains functional leucine-rich nuclear export signals that are essential for splicing. Genetics166, 53–65 (2004). ArticleCASPubMedPubMed CentralGoogle Scholar
- Tkacz, I. D. et al. Identification of novel snRNA-specific Sm proteins that bind selectively to U2 and U4 snRNAs in Trypanosoma brucei. RNA13, 30–43 (2007). ArticleCASPubMedPubMed CentralGoogle Scholar
- Palfi, Z. et al. SMN-assisted assembly of snRNP-specific Sm cores in trypanosomes. Genes Dev.23, 1650–1664 (2009). ArticleCASPubMedPubMed CentralGoogle Scholar
- Jae, N. et al. snRNA-specific role of SMN in trypanosome snRNP biogenesis in vivo. RNA Biol.8, 90–100 (2011). ArticleCASPubMedPubMed CentralGoogle Scholar
- Hernandez-Verdun, D., Roussel, P., Thiry, M., Sirri, V. & Lafontaine, D. L. The nucleolus: structure/function relationship in RNA metabolism. Wiley Interdiscip. Rev. RNA1, 415–431 (2010). ArticleCASPubMedGoogle Scholar
- Ohno, M. Size matters in RNA export. RNA Biol.9, 1413–1417 (2012). ArticleCASPubMedGoogle Scholar
- Cullen, B. R. Nuclear RNA export. J. Cell Sci.116, 587–597 (2003). ArticlePubMedGoogle Scholar
- Ohno, M., Segref, A., Kuersten, S. & Mattaj, I. W. Identity elements used in export of mRNAs. Mol. Cell9, 659–671 (2002). ArticleCASPubMedGoogle Scholar
- Masuyama, K., Taniguchi, I., Kataoka, N. & Ohno, M. RNA length defines RNA export pathway. Genes Dev.18, 2074–2085 (2004). ArticleCASPubMedPubMed CentralGoogle Scholar
- Fuke, H. & Ohno, M. Role of poly (A) tail as an identity element for mRNA nuclear export. Nucleic Acids Res.36, 1037–1049 (2008). ArticleCASPubMedGoogle Scholar
- McCloskey, A. Taniguchi, I., Shinmyozu, K. & Ohno, M. hnRNP C tetramer measures RNA length to classify RNA polymerase II transcripts for export. Science335, 1643–1646 (2012). First identification of a specific function for the non-shuttling hnRNP C-type proteins in RNA export.ArticleCASPubMedGoogle Scholar
- Izaurralde, E. et al. A nuclear cap binding protein complex involved in pre-mRNA splicing. Cell78, 657–668 (1994). ArticleCASPubMedGoogle Scholar
- Ohno, M., Segref, A., Bachi, A., Wilm, M. & Mattaj, I. W. PHAX, a mediator of U snRNA nuclear export whose activity is regulated by phosphorylation. Cell101, 187–198 (2000). ArticleCASPubMedGoogle Scholar
- Hallais, M. et al. CBC–ARS2 stimulates 3′-end maturation of multiple RNA families and favors cap-proximal processing. Nature Struct. Mol. Biol.20, 1358–1366 (2013). Shows that Ars2 forms 5′ cap-binding subcomplexes that participate in 3′-end processing of three distinct classes of transcript.ArticleCASGoogle Scholar
- Fornerod, M., Ohno, M., Yoshida, M. & Mattaj, I. W. CRM1 is an export receptor for leucine-rich nuclear export signals. Cell90, 1051–1060 (1997). ArticleCASPubMedGoogle Scholar
- Smith, K. P. & Lawrence, J. B. Interactions of U2 gene loci and their nuclear transcripts with Cajal (coiled) bodies: evidence for PreU2 within Cajal bodies. Mol. Biol. Cell11, 2987–2998 (2000). ArticleCASPubMedPubMed CentralGoogle Scholar
- Suzuki, T., Izumi, H. & Ohno, M. Cajal body surveillance of U snRNA export complex assembly. J. Cell Biol.190, 603–612 (2010). ArticleCASPubMedPubMed CentralGoogle Scholar
- Boulon, S. et al. PHAX and CRM1 are required sequentially to transport U3 snoRNA to nucleoli. Mol. Cell16, 777–787 (2004). ArticleCASPubMedGoogle Scholar
- Frey, M. R. & Matera, A. G. RNA-mediated interaction of Cajal bodies and U2 snRNA genes. J. Cell Biol.154, 499–509 (2001). ArticleCASPubMedPubMed CentralGoogle Scholar
- Lemm, I. et al. Ongoing U snRNP biogenesis is required for the integrity of Cajal bodies. Mol. Biol. Cell17, 3221–3231 (2006). ArticleCASPubMedPubMed CentralGoogle Scholar
- Matera, A. G., Izaguire-Sierra, M., Praveen, K. & Rajendra, T. K. Nuclear bodies: random aggregates of sticky proteins or crucibles of macromolecular assembly? Dev. Cell17, 639–647 (2009). ArticleCASPubMedPubMed CentralGoogle Scholar
- Kitao, S. et al. A compartmentalized phosphorylation/dephosphorylation system that regulates U snRNA export from the nucleus. Mol. Cell. Biol.28, 487–497 (2008). ArticleCASPubMedGoogle Scholar
- Meister, G., Buhler, D., Pillai, R., Lottspeich, F. & Fischer, U. A multiprotein complex mediates the ATP-dependent assembly of spliceosomal U snRNPs. Nature Cell Biol.3, 945–949 (2001). ArticleCASPubMedGoogle Scholar
- Pellizzoni, L., Yong, J. & Dreyfuss, G. Essential role for the SMN complex in the specificity of snRNP assembly. Science298, 1775–1779 (2002). ArticleCASPubMedGoogle Scholar
- Massenet, S., Pellizzoni, L., Paushkin, S., Mattaj, I. W. & Dreyfuss, G. The SMN complex is associated with snRNPs throughout their cytoplasmic assembly pathway. Mol. Cell. Biol.22, 6533–6541 (2002). ArticleCASPubMedPubMed CentralGoogle Scholar
- Narayanan, U., Ospina, J. K., Frey, M. R., Hebert, M. D. & Matera, A. G. SMN, the spinal muscular atrophy protein, forms a pre-import snRNP complex with snurportin1 and importin β. Hum. Mol. Genet.11, 1785–1795 (2002). ArticleCASPubMedGoogle Scholar
- Mouaikel, J. et al. Interaction between the small-nuclear-RNA cap hypermethylase and the spinal muscular atrophy protein, survival of motor neuron. EMBO Rep.4, 616–622 (2003). ArticleCASPubMedPubMed CentralGoogle Scholar
- Meister, G. et al. Methylation of Sm proteins by a complex containing PRMT5 and the putative U snRNP assembly factor pICln. Curr. Biol.11, 1990–1994 (2001). ArticleCASPubMedGoogle Scholar
- Friesen, W. J. et al. The methylosome, a 20S complex containing JBP1 and pICln, produces dimethylarginine-modified Sm proteins. Mol. Cell. Biol.21, 8289–8300 (2001). ArticleCASPubMedPubMed CentralGoogle Scholar
- Grimm, C. et al. Structural basis of assembly chaperone-mediated snRNP formation. Mol. Cell49, 692–703 (2013). ArticleCASPubMedGoogle Scholar
- Chari, A. et al. An assembly chaperone collaborates with the SMN complex to generate spliceosomal snRNPs. Cell135, 497–509 (2008). ArticleCASPubMedGoogle Scholar
- Yong, J., Kasim, M., Bachorik, J. L., Wan, L. & Dreyfuss, G. Gemin5 delivers snRNA precursors to the SMN complex for snRNP biogenesis. Mol. Cell38, 551–562 (2010). ArticleCASPubMedPubMed CentralGoogle Scholar
- Raker, V. A., Plessel, G. & Luhrmann, R. The snRNP core assembly pathway: identification of stable core protein heteromeric complexes and an snRNP subcore particle in vitro. EMBO J.15, 2256–2269 (1996). ArticleCASPubMedPubMed CentralGoogle Scholar
- Kambach, C. et al. Crystal structures of two Sm protein complexes and their implications for the assembly of the spliceosomal snRNPs. Cell96, 375–387 (1999). ArticleCASPubMedGoogle Scholar
- Leung, A. K., Nagai, K. & Li, J. Structure of the spliceosomal U4 snRNP core domain and its implication for snRNP biogenesis. Nature473, 536–539 (2011). Co-crystal structure of U4 snRNA construct with an Sm core definitively shows that the RNA passes through the hole in the Sm ring.ArticleCASPubMedPubMed CentralGoogle Scholar
- Kroiss, M. et al. Evolution of an RNP assembly system: a minimal SMN complex facilitates formation of UsnRNPs in Drosophila melanogaster. Proc. Natl Acad. Sci. USA105, 10045–10050 (2008). Shows that both human and fruitfly SMN–GEMIN2 heterodimers are sufficient for mediating Sm core assemblyin vitro. ArticleCASPubMedPubMed CentralGoogle Scholar
- Zhang, R. et al. Structure of a key intermediate of the SMN complex reveals Gemin2's crucial function in snRNP assembly. Cell146, 384–395 (2011). Together with reference 46, these papers identify key intermediates in the Sm core assembly pathway, highlighting an unexpected role for GEMIN2.ArticleCASPubMedPubMed CentralGoogle Scholar
- Liu, Q., Fischer, U., Wang, F. & Dreyfuss, G. The spinal muscular atrophy disease gene product, SMN, and its associated protein SIP1 are in a complex with spliceosomal snRNP proteins. Cell90, 1013–1021 (1997). ArticleCASPubMedGoogle Scholar
- Buhler, D., Raker, V., Luhrmann, R. & Fischer, U. Essential role for the tudor domain of SMN in spliceosomal U snRNP assembly: implications for spinal muscular atrophy. Hum. Mol. Genet.8, 2351–2357 (1999). ArticleCASPubMedGoogle Scholar
- Pellizzoni, L., Charroux, B. & Dreyfuss, G. SMN mutants of spinal muscular atrophy patients are defective in binding to snRNP proteins. Proc. Natl Acad. Sci. USA96, 11167–11172 (1999). ArticleCASPubMedPubMed CentralGoogle Scholar
- Hannus, S., Buhler, D., Romano, M., Seraphin, B. & Fischer, U. The Schizosaccharomyces pombe protein Yab8p and a novel factor, Yip1p, share structural and functional similarity with the spinal muscular atrophy-associated proteins SMN and SIP1. Hum. Mol. Genet.9, 663–674 (2000). ArticleCASPubMedGoogle Scholar
- Rajendra, T. K. et al. A Drosophila melanogaster model of spinal muscular atrophy reveals a function for SMN in striated muscle. J. Cell Biol.176, 831–841 (2007). ArticleCASPubMedPubMed CentralGoogle Scholar
- Shpargel, K. B. & Matera, A. G. Gemin proteins are required for efficient assembly of Sm-class ribonucleoproteins. Proc. Natl Acad. Sci. USA102, 17372–17377 (2005). Assays individual Gemins, as well as a panel of SMN missense mutants for ability to carry out Sm core assembly, showing that certain SMA-causing alleles are functional, whereas others are not.ArticleCASPubMedPubMed CentralGoogle Scholar
- Selenko, P. et al. SMN Tudor domain structure and its interaction with the Sm proteins. Nature Struct. Biol.8, 27–31 (2001). ArticleCASPubMedGoogle Scholar
- Lorson, C. L. et al. SMN oligomerization defect correlates with spinal muscular atrophy severity. Nature Genet.19, 63–66 (1998). ArticleCASPubMedGoogle Scholar
- Martin, R., Gupta, K., Ninan, N. S., Perry, K. & Van Duyne, G. D. The survival motor neuron protein forms soluble glycine zipper oligomers. Structure20, 1929–1939 (2012). ArticleCASPubMedPubMed CentralGoogle Scholar
- Fischer, U. & Luhrmann, R. An essential signaling role for the m3G cap in the transport of U1 snRNP to the nucleus. Science249, 786–790 (1990). ArticleCASPubMedGoogle Scholar
- Narayanan, U., Achsel, T., Luhrmann, R. & Matera, A. G. Coupled in vitro import of U snRNPs and SMN, the spinal muscular atrophy protein. Mol. Cell16, 223–234 (2004). ArticleCASPubMedGoogle Scholar
- Fischer, U., Sumpter, V., Sekine, M., Satoh, T. & Luhrmann, R. Nucleo-cytoplasmic transport of U snRNPs: definition of a nuclear location signal in the Sm core domain that binds a transport receptor independently of the m3G cap. EMBO J.12, 573–583 (1993). ArticleCASPubMedPubMed CentralGoogle Scholar
- Huber, J. et al. Snurportin1, an m3G-cap-specific nuclear import receptor with a novel domain structure. EMBO J.17, 4114–4126 (1998). ArticleCASPubMedPubMed CentralGoogle Scholar
- Palacios, I., Hetzer, M., Adam, S. A. & Mattaj, I. W. Nuclear import of U snRNPs requires importin β. EMBO J.16, 6783–6792 (1997). ArticleCASPubMedPubMed CentralGoogle Scholar
- Fischer, U., Liu, Q. & Dreyfuss, G. The SMN–SIP1 complex has an essential role in spliceosomal snRNP biogenesis. Cell90, 1023–1029 (1997). ArticleCASPubMedGoogle Scholar
- Neubauer, G. et al. Mass spectrometry and EST-database searching allows characterization of the multi-protein spliceosome complex. Nature Genet.20, 46–50 (1998). ArticleCASPubMedGoogle Scholar
- Trinkle-Mulcahy, L. et al. Identifying specific protein interaction partners using quantitative mass spectrometry and bead proteomes. J. Cell Biol.183, 223–239 (2008). ArticleCASPubMedPubMed CentralGoogle Scholar
- Herold, N. et al. Conservation of the protein composition and electron microscopy structure of Drosophila melanogaster and human spliceosomal complexes. Mol. Cell. Biol.29, 281–301 (2009). ArticleCASPubMedGoogle Scholar
- Matera, A. G. & Shpargel, K. B. Pumping RNA: nuclear bodybuilding along the RNP pipeline. Curr. Opin. Cell Biol.18, 317–324 (2006). ArticleCASPubMedGoogle Scholar
- Stanek, D. & Neugebauer, K. M. The Cajal body: a meeting place for spliceosomal snRNPs in the nuclear maze. Chromosoma115, 343–354 (2006). ArticleCASPubMedGoogle Scholar
- Sleeman, J. E. & Lamond, A. I. Newly assembled snRNPs associate with coiled bodies before speckles, suggesting a nuclear snRNP maturation pathway. Curr. Biol.9, 1065–1074 (1999). ArticleCASPubMedGoogle Scholar
- Lamond, A. I. & Spector, D. L. Nuclear speckles: a model for nuclear organelles. Nature Rev. Mol. Cell Biol.4, 605–612 (2003). ArticleCASGoogle Scholar
- Ospina, J. K. et al. Cross-talk between snurportin1 subdomains. Mol. Biol. Cell16, 4660–4671 (2005). ArticleCASPubMedPubMed CentralGoogle Scholar
- Jady, B. E. et al. Modification of Sm small nuclear RNAs occurs in the nucleoplasmic Cajal body following import from the cytoplasm. EMBO J.22, 1878–1888 (2003). ArticleCASPubMedPubMed CentralGoogle Scholar
- Nesic, D., Tanackovic, G. & Kramer, A. A role for Cajal bodies in the final steps of U2 snRNP biogenesis. J. Cell Sci.117, 4423–4433 (2004). ArticleCASPubMedGoogle Scholar
- Schaffert, N., Hossbach, M., Heintzmann, R., Achsel, T. & Luhrmann, R. RNAi knockdown of hPrp31 leads to an accumulation of U4/U6 di-snRNPs in Cajal bodies. EMBO J.23, 3000–3009 (2004). ArticleCASPubMedPubMed CentralGoogle Scholar
- Novotny, I., Blazikova, M., Stanek, D., Herman, P. & Malinsky, J. In vivo kinetics of U4/U6. U5 tri-snRNP formation in Cajal bodies. Mol. Biol. Cell22, 513–523 (2011). ArticleCASPubMedPubMed CentralGoogle Scholar
- Stanek, D. & Neugebauer, K. M. Detection of snRNP assembly intermediates in Cajal bodies by fluorescence resonance energy transfer. J. Cell Biol.166, 1015–1025 (2004). ArticleCASPubMedPubMed CentralGoogle Scholar
- Stanek, D., Rader, S. D., Klingauf, M. & Neugebauer, K. M. Targeting of U4/U6 small nuclear RNP assembly factor SART3/p110 to Cajal bodies. J. Cell Biol.160, 505–516 (2003). ArticleCASPubMedPubMed CentralGoogle Scholar
- Strzelecka, M., Oates, A. C. & Neugebauer, K. M. Dynamic control of Cajal body number during zebrafish embryogenesis. Nucleus1, 96–108 (2010). ArticlePubMedPubMed CentralGoogle Scholar
- Takata, H., Nishijima, H., Maeshima, K. & Shibahara, K. The integrator complex is required for integrity of Cajal bodies. J. Cell Sci.125, 166–175 (2012). ArticleCASPubMedGoogle Scholar
- Tucker, K. E. et al. Residual Cajal bodies in coilin knockout mice fail to recruit Sm snRNPs and SMN, the spinal muscular atrophy gene product. J. Cell Biol.154, 293–307 (2001). ArticleCASPubMedPubMed CentralGoogle Scholar
- Liu, J. L. et al. Coilin is essential for Cajal body organization in Drosophila melanogaster. Mol. Biol. Cell20, 1661–1670 (2009). ArticleCASPubMedPubMed CentralGoogle Scholar
- Walker, M. P., Tian, L. & Matera, A. G. Reduced viability, fertility and fecundity in mice lacking the cajal body marker protein, coilin. PLoS ONE4, e6171 (2009). ArticlePubMedPubMed CentralCASGoogle Scholar
- Strzelecka, M. et al. Coilin-dependent snRNP assembly is essential for zebrafish embryogenesis. Nature Struct. Mol. Biol.17, 403–409 (2010). ArticleCASGoogle Scholar
- Spector, D. L. & Lamond, A. I. Nuclear speckles. Cold Spring Harb. Perspect. Biol.3, a000646 (2011). ArticlePubMedPubMed CentralCASGoogle Scholar
- Hall, L. L., Smith, K. P., Byron, M. & Lawrence, J. B. Molecular anatomy of a speckle. Anat. Rec. A Discov. Mol. Cell. Evol. Biol.288, 664–675 (2006). ArticlePubMedPubMed CentralGoogle Scholar
- Girard, C. et al. Post-transcriptional spliceosomes are retained in nuclear speckles until splicing completion. Nature Commun.3, 994 (2012). ArticleCASGoogle Scholar
- Valadkhan, S. Role of the snRNAs in spliceosomal active site. RNA Biol.7, 345–353 (2010). ArticleCASPubMedGoogle Scholar
- Du, H. & Rosbash, M. The U1 snRNP protein U1C recognizes the 5′ splice site in the absence of base pairing. Nature419, 86–90 (2002). ArticleCASPubMedGoogle Scholar
- Wiesner, S., Stier, G., Sattler, M. & Macias, M. J. Solution structure and ligand recognition of the WW domain pair of the yeast splicing factor Prp40. J. Mol. Biol.324, 807–822 (2002). ArticleCASPubMedGoogle Scholar
- Morris, D. P. & Greenleaf, A. L. The splicing factor, Prp40, binds the phosphorylated carboxyl-terminal domain of RNA polymerase II. J. Biol. Chem.275, 39935–39943 (2000). ArticleCASPubMedGoogle Scholar
- Gornemann, J. et al. Cotranscriptional spliceosome assembly and splicing are independent of the Prp40p WW domain. RNA17, 2119–2129 (2011). ArticlePubMedPubMed CentralCASGoogle Scholar
- Staknis, D. & Reed, R. SR proteins promote the first specific recognition of Pre-mRNA and are present together with the U1 small nuclear ribonucleoprotein particle in a general splicing enhancer complex. Mol. Cell. Biol.14, 7670–7682 (1994). ArticleCASPubMedPubMed CentralGoogle Scholar
- Cho, S. et al. Interaction between the RNA binding domains of Ser-Arg splicing factor 1 and U1–70K snRNP protein determines early spliceosome assembly. Proc. Natl Acad. Sci. USA108, 8233–8238 (2011). ArticleCASPubMedPubMed CentralGoogle Scholar
- Pabis, M. et al. The nuclear cap-binding complex interacts with the U4/U6. U5 tri-snRNP and promotes spliceosome assembly in mammalian cells. RNA19, 1054–1063 (2013). ArticleCASPubMedPubMed CentralGoogle Scholar
- Fox-Walsh, K. L. et al. The architecture of pre-mRNAs affects mechanisms of splice-site pairing. Proc. Natl Acad. Sci. USA102, 16176–16181 (2005). ArticleCASPubMedPubMed CentralGoogle Scholar
- Xiao, X., Wang, Z., Jang, M. & Burge, C. B. Coevolutionary networks of splicing cis-regulatory elements. Proc. Natl Acad. Sci. USA104, 18583–18588 (2007). ArticleCASPubMedPubMed CentralGoogle Scholar
- Sterner, D. A., Carlo, T. & Berget, S. M. Architectural limits on split genes. Proc. Natl Acad. Sci. USA93, 15081–15085 (1996). ArticleCASPubMedPubMed CentralGoogle Scholar
- De Conti, L., Baralle, M. & Buratti, E. Exon and intron definition in pre-mRNA splicing. Wiley Interdiscip. Rev. RNA4, 49–60 (2013). ArticleCASPubMedGoogle Scholar
- Bonnal, S. et al. RBM5/Luca-15/H37 regulates Fas alternative splice site pairing after exon definition. Mol. Cell32, 81–95 (2008). ArticleCASPubMedGoogle Scholar
- Sharma, S., Kohlstaedt, L. A., Damianov, A., Rio, D. C. & Black, D. L. Polypyrimidine tract binding protein controls the transition from exon definition to an intron defined spliceosome. Nature Struct. Mol. Biol.15, 183–191 (2008). Demonstrates that an early step in spliceosome assembly (transition from exon definition to intron definition complex) is a key stage for splicing regulation.ArticleCASGoogle Scholar
- Sun, J. S. & Manley, J. L. A novel U2–U6 snRNA structure is necessary for mammalian mRNA splicing. Genes Dev.9, 843–854 (1995). ArticleCASPubMedGoogle Scholar
- Raghunathan, P. L. & Guthrie, C. RNA unwinding in U4/U6 snRNPs requires ATP hydrolysis and the DEIH-box splicing factor Brr2. Curr. Biol.8, 847–855 (1998). ArticleCASPubMedGoogle Scholar
- Ilagan, J. O., Chalkley, R. J., Burlingame, A. L. & Jurica, M. S. Rearrangements within human spliceosomes captured after exon ligation. RNA19, 400–412 (2013) ArticleCASPubMedPubMed CentralGoogle Scholar
- Schwer, B. & Gross, C. H. Prp22, a DExH-box RNA helicase, plays two distinct roles in yeast pre-mRNA splicing. EMBO J.17, 2086–2094 (1998). ArticleCASPubMedPubMed CentralGoogle Scholar
- Fourmann, J. B. et al. Dissection of the factor requirements for spliceosome disassembly and the elucidation of its dissociation products using a purified splicing system. Genes Dev.27, 413–428 (2013). ArticleCASPubMedPubMed CentralGoogle Scholar
- Abelson, J. et al. Conformational dynamics of single pre-mRNA molecules during in vitro splicing. Nature Struct. Mol. Biol.17, 504–512 (2010). ArticleCASGoogle Scholar
- Hoskins, A. A. et al. Ordered and dynamic assembly of single spliceosomes. Science331, 1289–1295 (2011). ArticleCASPubMedPubMed CentralGoogle Scholar
- Tseng, C. K. & Cheng, S. C. Both catalytic steps of nuclear pre-mRNA splicing are reversible. Science320, 1782–1784 (2008). ArticleCASPubMedGoogle Scholar
- Yang, F. et al. Splicing proofreading at 5′ splice sites by ATPase Prp28p. Nucleic Acids Res.41, 4660–4670 (2013). ArticleCASPubMedPubMed CentralGoogle Scholar
- Malca, H., Shomron, N. & Ast, G. The U1 snRNP base pairs with the 5′ splice site within a penta–snRNP complex. Mol. Cell. Biol.23, 3442–3455 (2003). ArticleCASPubMedPubMed CentralGoogle Scholar
- Stevens, S. W. et al. Composition and functional characterization of the yeast spliceosomal penta–snRNP. Mol. Cell9, 31–44 (2002). ArticleCASPubMedGoogle Scholar
- Gornemann, J., Kotovic, K. M., Hujer, K. & Neugebauer, K. M. Cotranscriptional spliceosome assembly occurs in a stepwise fashion and requires the cap binding complex. Mol. Cell19, 53–63 (2005). Development of a novel chromatin immunoprecipitation assay to investigate co-transcriptional spliceosome assembly, demonstrating a role for the CBC in recruitment of snRNPs to nascent pre-mRNA transcripts.ArticlePubMedCASGoogle Scholar
- Behzadnia, N., Hartmuth, K., Will, C. L. & Luhrmann, R. Functional spliceosomal A complexes can be assembled in vitro in the absence of a penta–snRNP. RNA12, 1738–1746 (2006). ArticleCASPubMedPubMed CentralGoogle Scholar
- Schneider, M. et al. Exon definition complexes contain the tri-snRNP and can be directly converted into B-like precatalytic splicing complexes. Mol. Cell38, 223–235 (2010). Together with reference 116, these studies suggest the existence of alternative spliceosome assembly pathways.ArticleCASPubMedGoogle Scholar
- Madhani, H. D. & Guthrie, C. Dynamic RNA–RNA interactions in the spliceosome. Annu. Rev. Genet.28, 1–26 (1994). ArticleCASPubMedGoogle Scholar
- Valadkhan, S., Mohammadi, A., Wachtel, C. & Manley, J. L. Protein-free spliceosomal snRNAs catalyze a reaction that resembles the first step of splicing. RNA13, 2300–2311 (2007). ArticleCASPubMedPubMed CentralGoogle Scholar
- Valadkhan, S., Mohammadi, A., Jaladat, Y. & Geisler, S. Protein-free small nuclear RNAs catalyze a two-step splicing reaction. Proc. Natl Acad. Sci. USA106, 11901–11906 (2009). Together with reference 122, demonstrates that protein-free U6/U2 snRNA constructs can recognize 5′ splice site and branch point sequence to carry out the first and second steps of splicing.ArticleCASPubMedPubMed CentralGoogle Scholar
- Marcia, M. & Pyle, A. M. Visualizing group II intron catalysis through the stages of splicing. Cell151, 497–507 (2012). ArticleCASPubMedPubMed CentralGoogle Scholar
- Toor, N., Keating, K. S. & Pyle, A. M. Structural insights into RNA splicing. Curr. Opin. Struct. Biol.19, 260–266 (2009). ArticleCASPubMedPubMed CentralGoogle Scholar
- Toor, N., Keating, K. S., Taylor, S. D. & Pyle, A. M. Crystal structure of a self-spliced group II intron. Science320, 77–82 (2008). ArticleCASPubMedPubMed CentralGoogle Scholar
- Fica, S. M. et al. RNA catalyses nuclear pre-mRNA splicing. Nature503, 229–234 (2013). ArticleCASPubMedPubMed CentralGoogle Scholar
- Butcher, S. E. The spliceosome and its metal ions. Met. Ions Life Sci.9, 235–251 (2011). ArticleCASPubMedGoogle Scholar
- Cordin, O., Hahn, D. & Beggs, J. D. Structure, function and regulation of spliceosomal RNA helicases. Curr. Opin. Cell Biol.24, 431–438 (2012). ArticleCASPubMedGoogle Scholar
- Small, E. C., Leggett, S. R., Winans, A. A. & Staley, J. P. The EF-G-like GTPase Snu114p regulates spliceosome dynamics mediated by Brr2p, a DExD/H box ATPase. Mol. Cell23, 389–399 (2006). ArticleCASPubMedPubMed CentralGoogle Scholar
- Galej, W. P., Oubridge, C., Newman, A. J. & Nagai, K. Crystal structure of Prp8 reveals active site cavity of the spliceosome. Nature493, 638–643 (2013). ArticleCASPubMedPubMed CentralGoogle Scholar
- Schellenberg, M. J. et al. A conformational switch in PRP8 mediates metal ion coordination that promotes pre-mRNA exon ligation. Nature Struct. Mol. Biol.20, 728–734 (2013). ArticleCASGoogle Scholar
- Mozaffari-Jovin, S. et al. Inhibition of RNA helicase Brr2 by the C-terminal tail of the spliceosomal protein Prp8. Science341, 80–84 (2013). ArticleCASPubMedGoogle Scholar
- Ohrt, T. et al. Molecular dissection of step 2 catalysis of yeast pre-mRNA splicing investigated in a purified system. RNA19, 902–915 (2013). ArticleCASPubMedPubMed CentralGoogle Scholar
- Sun, H. & Chasin, L. A. Multiple splicing defects in an intronic false exon. Mol. Cell. Biol.20, 6414–6425 (2000). ArticleCASPubMedPubMed CentralGoogle Scholar
- Matlin, A. J., Clark, F. & Smith, C. W. Understanding alternative splicing: towards a cellular code. Nature Rev. Mol. Cell Biol.6, 386–398 (2005). ArticleCASGoogle Scholar
- Wang, Z. & Burge, C. B. Splicing regulation: from a parts list of regulatory elements to an integrated splicing code. RNA14, 802–813 (2008). ArticleCASPubMedPubMed CentralGoogle Scholar
- Bessonov, S., Anokhina, M., Will, C. L., Urlaub, H. & Luhrmann, R. Isolation of an active step I spliceosome and composition of its RNP core. Nature452, 846–850 (2008). ArticleCASPubMedGoogle Scholar
- Zhou, Z., Licklider, L. J., Gygi, S. P. & Reed, R. Comprehensive proteomic analysis of the human spliceosome. Nature419, 182–185 (2002). Identifies more than 100 proteins in the active spliceosome, many more than the known protein components of snRNPs.ArticleCASPubMedGoogle Scholar
- Hegele, A. et al. Dynamic protein–protein interaction wiring of the human spliceosome. Mol. Cell45, 567–580 (2012). ArticleCASPubMedGoogle Scholar
- Izquierdo, J. M. et al. Regulation of Fas alternative splicing by antagonistic effects of TIA-1 and PTB on exon definition. Mol. Cell19, 475–484 (2005). ArticleCASPubMedGoogle Scholar
- Sharma, S., Maris, C., Allain, F. H. & Black, D. L. U1 snRNA directly interacts with polypyrimidine tract-binding protein during splicing repression. Mol. Cell41, 579–588 (2011). ArticleCASPubMedPubMed CentralGoogle Scholar
- Chiou, N. T., Shankarling, G. & Lynch, K. W. HnRNP L and hnRNP A1 induce extended U1 snRNA interactions with an exon to repress spliceosome assembly. Mol. Cell49, 972–982 (2013). ArticleCASPubMedPubMed CentralGoogle Scholar
- House, A. E. & Lynch, K. W. An exonic splicing silencer represses spliceosome assembly after ATP-dependent exon recognition. Nature Struct. Mol. Biol.13, 937–944 (2006). ArticleCASGoogle Scholar
- McCullough, A. J. & Berget, S. M. G triplets located throughout a class of small vertebrate introns enforce intron borders and regulate splice site selection. Mol. Cell. Biol.17, 4562–4571 (1997). ArticleCASPubMedPubMed CentralGoogle Scholar
- Chou, M. Y., Rooke, N., Turck, C. W. & Black, D. L. hnRNP H is a component of a splicing enhancer complex that activates a c-src alternative exon in neuronal cells. Mol. Cell. Biol.19, 69–77 (1999). ArticleCASPubMedPubMed CentralGoogle Scholar
- Chen, C. D., Kobayashi, R. & Helfman, D. M. Binding of hnRNP H to an exonic splicing silencer is involved in the regulation of alternative splicing of the rat β-tropomyosin gene. Genes Dev.13, 593–606 (1999). ArticleCASPubMedPubMed CentralGoogle Scholar
- Caputi, M. & Zahler, A. M. Determination of the RNA binding specificity of the heterogeneous nuclear ribonucleoprotein (hnRNP) H/H′/F/2H9 family. J. Biol. Chem.276, 43850–43859 (2001). ArticleCASPubMedGoogle Scholar
- Ule, J. et al. An RNA map predicting Nova-dependent splicing regulation. Nature444, 580–586 (2006). ArticleCASPubMedGoogle Scholar
- Wang, Y. et al. A complex network of factors with overlapping affinities represses splicing through intronic elements. Nature Struct. Mol. Biol.20, 36–45 (2013). Suggests that interactions between variouscis-acting elements andtrans-acting factors form a complex network that controls context-dependent splicing.ArticleCASGoogle Scholar
- Borah, S., Wong, A. C. & Steitz, J. A. Drosophila hnRNP A1 homologs Hrp36/Hrp38 enhance U2-type versus U12-type splicing to regulate alternative splicing of the prospero twintron. Proc. Natl Acad. Sci. USA106, 2577–2582 (2009). ArticleCASPubMedPubMed CentralGoogle Scholar
- Wang, Z. et al. Systematic identification and analysis of exonic splicing silencers. Cell119, 831–845 (2004). ArticleCASPubMedGoogle Scholar
- Yu, Y. et al. Dynamic regulation of alternative splicing by silencers that modulate 5′ splice site competition. Cell135, 1224–1236 (2008). ArticleCASPubMedPubMed CentralGoogle Scholar
- Donahue, C. P., Muratore, C., Wu, J. Y., Kosik, K. S. & Wolfe, M. S. Stabilization of the tau exon 10 stem loop alters pre-mRNA splicing. J. Biol. Chem.281, 23302–23306 (2006). ArticleCASPubMedGoogle Scholar
- Graveley, B. R. Mutually exclusive splicing of the insect Dscam pre-mRNA directed by competing intronic RNA secondary structures. Cell123, 65–73 (2005). A great example of how RNA structures can have a leading role in controlling a complicated regimen of mutally exclusive splicing.ArticleCASPubMedPubMed CentralGoogle Scholar
- Yang, Y. et al. RNA secondary structure in mutually exclusive splicing. Nature Struct. Mol. Biol.18, 159–168 (2011). ArticleCASGoogle Scholar
- Wang, X. et al. An RNA architectural locus control region involved in Dscam mutually exclusive splicing. Nature Commun.3, 1255 (2012). ArticleCASGoogle Scholar
- Bleichert, F. & Baserga, S. J. The long unwinding road of RNA helicases. Mol. Cell27, 339–352 (2007). ArticleCASPubMedGoogle Scholar
- Honig, A., Auboeuf, D., Parker, M. M., O'Malley, B. W. & Berget, S. M. Regulation of alternative splicing by the ATP-dependent DEAD-box RNA helicase p72. Mol. Cell. Biol.22, 5698–5707 (2002). ArticleCASPubMedPubMed CentralGoogle Scholar
- Lee, C. G. RH70, a bidirectional RNA helicase, co-purifies with U1snRNP. J. Biol. Chem.277, 39679–39683 (2002). ArticleCASPubMedGoogle Scholar
- Weeks, K. M. Advances in RNA structure analysis by chemical probing. Curr. Opin. Struct. Biol.20, 295–304 (2010). ArticleCASPubMedPubMed CentralGoogle Scholar
- Khodor, Y. L. et al. Nascent-seq indicates widespread cotranscriptional pre-mRNA splicing in Drosophila. Genes Dev.25, 2502–2512 (2011). ArticleCASPubMedPubMed CentralGoogle Scholar
- Ip, J. Y. et al. Global impact of RNA polymerase II elongation inhibition on alternative splicing regulation. Genome Res.21, 390–401 (2011). ArticleCASPubMedPubMed CentralGoogle Scholar
- Roberts, G. C., Gooding, C., Mak, H. Y., Proudfoot, N. J. & Smith, C. W. Co-transcriptional commitment to alternative splice site selection. Nucleic Acids Res.26, 5568–5572 (1998). ArticleCASPubMedPubMed CentralGoogle Scholar
- Kornblihtt, A. R. et al. Alternative splicing: a pivotal step between eukaryotic transcription and translation. Nature Rev. Mol. Cell Biol.14, 153–165 (2013). ArticleCASGoogle Scholar
- Brugiolo, M., Herzel, L. & Neugebauer, K. M. Counting on co-transcriptional splicing. F1000Prime Rep.5, 9 (2013). ArticlePubMedPubMed CentralGoogle Scholar
- Wang, Y., Ma, M., Xiao, X. & Wang, Z. Intronic splicing enhancers, cognate splicing factors and context-dependent regulation rules. Nature Struct. Mol. Biol.19, 1044–1052 (2012). ArticleCASGoogle Scholar
- Spellman, R., Llorian, M. & Smith, C. W. Crossregulation and functional redundancy between the splicing regulator PTB and its paralogs nPTB and ROD1. Mol. Cell27, 420–434 (2007). ArticleCASPubMedPubMed CentralGoogle Scholar
- Boutz, P. L. et al. A post-transcriptional regulatory switch in polypyrimidine tract-binding proteins reprograms alternative splicing in developing neurons. Genes Dev.21, 1636–1652 (2007). ArticleCASPubMedPubMed CentralGoogle Scholar
- Barash, Y. et al. Deciphering the splicing code. Nature465, 53–59 (2010). ArticleCASPubMedGoogle Scholar
- Nilsen, T. W. & Graveley, B. R. Expansion of the eukaryotic proteome by alternative splicing. Nature463, 457–463 (2010). ArticleCASPubMedPubMed CentralGoogle Scholar
- Nilsen, T. W. The spliceosome: the most complex macromolecular machine in the cell? BioEssays25, 1147–1149 (2003). ArticlePubMedGoogle Scholar
- Singh, R. K. & Cooper, T. A. Pre-mRNA splicing in disease and therapeutics. Trends Mol. Med.18, 472–482 (2012). ArticleCASPubMedPubMed CentralGoogle Scholar
- Padgett, R. A. New connections between splicing and human disease. Trends Genet.28, 147–154 (2012). ArticleCASPubMedPubMed CentralGoogle Scholar
- Tanackovic, G. et al. PRPF mutations are associated with generalized defects in spliceosome formation and pre-mRNA splicing in patients with retinitis pigmentosa. Hum. Mol. Genet.20, 2116–2130 (2011). ArticleCASPubMedPubMed CentralGoogle Scholar
- Utz, V. M., Beight, C. D., Marino, M. J., Hagstrom, S. A. & Traboulsi, E. I. Autosomal dominant retinitis pigmentosa secondary to pre-mRNA splicing-factor gene PRPF31 (RP11): review of disease mechanism and report of a family with a novel 3-base pair insertion. Ophthalm. Genet.34, 183–188 (2013). ArticleCASGoogle Scholar
- Pena, V., Liu, S., Bujnicki, J. M., Luhrmann, R. & Wahl, M. C. Structure of a multipartite protein–protein interaction domain in splicing factor prp8 and its link to retinitis pigmentosa. Mol. Cell25, 615–624 (2007). ArticleCASPubMedGoogle Scholar
- He, H. et al. Mutations in U4atac snRNA, a component of the minor spliceosome, in the developmental disorder MOPD I. Science332, 238–240 (2011). ArticleCASPubMedPubMed CentralGoogle Scholar
- Lorson, C. L., Hahnen, E., Androphy, E. J. & Wirth, B. A single nucleotide in the SMN gene regulates splicing and is responsible for spinal muscular atrophy. Proc. Natl Acad. Sci. USA96, 6307–6311 (1999). ArticleCASPubMedPubMed CentralGoogle Scholar
- Schrank, B. et al. Inactivation of the survival motor neuron gene, a candidate gene for human spinal muscular atrophy, leads to massive cell death in early mouse embryos. Proc. Natl Acad. Sci. USA94, 9920–9925 (1997). ArticleCASPubMedPubMed CentralGoogle Scholar
- Gabanella, F. et al. Ribonucleoprotein assembly defects correlate with spinal muscular atrophy severity and preferentially affect a subset of spliceosomal snRNPs. PLoS ONE2, e921 (2007). ArticlePubMedPubMed CentralCASGoogle Scholar
- Praveen, K., Wen, Y. & Matera, A. G. A. Drosophila model of spinal muscular atrophy uncouples snRNP biogenesis functions of survival motor neuron from locomotion and viability defects. Cell Rep.1, 624–631 (2012). ArticleCASPubMedPubMed CentralGoogle Scholar
- Garcia, E. L., Lu, Z., Meers, M. P., Praveen, K. & Matera, A. G. Developmental arrest of Drosophila survival motor neuron (Smn) mutants accounts for differences in expression of minor intron-containing genes. RNA19, 1510–1516 (2013). ArticleCASPubMedPubMed CentralGoogle Scholar
- Baumer, D. et al. Alternative splicing events are a late feature of pathology in a mouse model of spinal muscular atrophy. PLoS Genet.5, e1000773 (2009). Together with references 182 and 183, these studies show that SMA phenotypes can be uncoupled from global splicing deficits. Using a missense allele that is active in Sm core assembly, reference 184 reveals a separation of SMN functions.ArticlePubMedPubMed CentralCASGoogle Scholar
- Cazzola, M., Rossi, M. & Malcovati, L. Biologic and clinical significance of somatic mutations of SF3B1 in myeloid and lymphoid neoplasms. Blood121, 260–269 (2013). ArticleCASPubMedPubMed CentralGoogle Scholar
- Yoshida, K. et al. Frequent pathway mutations of splicing machinery in myelodysplasia. Nature478, 64–69 (2011). ArticleCASPubMedGoogle Scholar
- Chesnais, V. et al. Spliceosome mutations in myelodysplastic syndromes and chronic myelomonocytic leukemia. Oncotarget3, 1284–1293 (2012). ArticlePubMedPubMed CentralGoogle Scholar
- Dhir, A., Buratti, E., van Santen, M. A., Luhrmann, R. & Baralle, F. E. The intronic splicing code: multiple factors involved in ATM pseudoexon definition. EMBO J.29, 749–760 (2010). ArticleCASPubMedPubMed CentralGoogle Scholar
- Lewandowska, M. A., Stuani, C., Parvizpur, A., Baralle, F. E. & Pagani, F. Functional studies on the ATM intronic splicing processing element. Nucleic Acids Res.33, 4007–4015 (2005). ArticleCASPubMedPubMed CentralGoogle Scholar
- Pagani, F. et al. A new type of mutation causes a splicing defect in ATM. Nature Genet.30, 426–429 (2002). ArticleCASPubMedGoogle Scholar
- Gunderson, S. I., Polycarpou-Schwarz, M. & Mattaj, I. W. U1 snRNP inhibits pre-mRNA polyadenylation through a direct interaction between U1 70K and poly(A) polymerase. Mol. Cell1, 255–264 (1998). ArticleCASPubMedGoogle Scholar
- Langemeier, J., Radtke, M. & Bohne, J. U1 snRNP-mediated poly(A) site suppression: beneficial and deleterious for mRNA fate. RNA Biol.10, 180–184 (2013). ArticleCASPubMedPubMed CentralGoogle Scholar
- Kaida, D. et al. U1 snRNP protects pre-mRNAs from premature cleavage and polyadenylation. Nature468, 664–668 (2010). ArticleCASPubMedPubMed CentralGoogle Scholar
- Almada, A. E., Wu, X., Kriz, A. J., Burge, C. B. & Sharp, P. A. Promoter directionality is controlled by U1 snRNP and polyadenylation signals. Nature499, 360–363 (2013). ArticleCASPubMedPubMed CentralGoogle Scholar
- Berg, M. G. et al. U1 snRNP determines mRNA length and regulates isoform expression. Cell150, 53–64 (2012). Together with reference 193, these genome-wide analyses illustrate a pervasive, non-splicing role for U1 snRNP in selection of the site of pre-mRNA 3′-end cleavage and polyadenylation.ArticleCASPubMedPubMed CentralGoogle Scholar
- Peterson, M. L., Bingham, G. L. & Cowan, C. Multiple features contribute to the use of the immunoglobulin M secretion-specific poly(A) signal but are not required for developmental regulation. Mol. Cell. Biol.26, 6762–6771 (2006). ArticleCASPubMedPubMed CentralGoogle Scholar
- Hall-Pogar, T., Liang, S., Hague, L. K. & Lutz, C. S. Specific trans-acting proteins interact with auxiliary RNA polyadenylation elements in the COX-2 3′-UTR. RNA13, 1103–1115 (2007). ArticleCASPubMedPubMed CentralGoogle Scholar
- Luo, W. et al. The conserved intronic cleavage and polyadenylation site of CstF-77 gene imparts control of 3′ end processing activity through feedback autoregulation and by U1 snRNP. PLoS Genet.9, e1003613 (2013). ArticleCASPubMedPubMed CentralGoogle Scholar
- Michaeli, S. Trans-splicing in trypanosomes: machinery and its impact on the parasite transcriptome. Future Microbiol.6, 459–474 (2011). ArticleCASPubMedGoogle Scholar
- Lasda, E. L. & Blumenthal, T. Trans-splicing. Wiley Interdiscip. Rev. RNA2, 417–434 (2011). ArticleCASPubMedGoogle Scholar
- Bruzik, J. P. & Maniatis, T. Spliced leader RNAs from lower eukaryotes are trans-spliced in mammalian cells. Nature360, 692–695 (1992). ArticleCASPubMedGoogle Scholar
- Smith, E. R. et al. The little elongation complex regulates small nuclear RNA transcription. Mol. Cell44, 954–965 (2011). ArticleCASPubMedPubMed CentralGoogle Scholar
- Fabrizio, P. et al. The evolutionarily conserved core design of the catalytic activation step of the yeast spliceosome. Mol. Cell36, 593–608 (2009). ArticleCASPubMedGoogle Scholar
Acknowledgements
Research in the authors' laboratories is supported by US National Institutes of Health grants R01-GM053034 and R01-NS041617 (to A.G.M.), as well as R01-CA158283 and R21-AR061640 (to Z.W.). The authors apologize to those whose work could not be discussed owing to space limitations.
Author information
Authors and Affiliations
- Department of Biology, Department of Genetics and Integrative Program for Biological and Genome Sciences, Lineberger Comprehensive Cancer Center, University of North Carolina, Chapel Hill, 27599, North Carolina, USA A. Gregory Matera
- Department of Pharmacology, Lineberger Comprehensive Cancer Center, University of North Carolina, Chapel Hill, 27599, North Carolina, USA Zefeng Wang
- A. Gregory Matera